From IHO by Larry Mayer
There is growing recognition that key to addressing critical issues like climate change, global sea level rise and the long-term sustainability of humankind is a more complete understanding of our oceans and processes within them that account for the distribution of global heat, CO and provide sustenance to so many.
Yet, despite years of effort, less than 25% of the global ocean seafloor has been mapped and less than 5% of the ocean volume explored, likely due to the cost and inefficiency of traditional ocean mapping and exploration techniques using large, very expensive, crewed research vessels.
Recent advances in the development of uncrewed surface vessels offer the possibility to reduce costs and increase efficiency of ocean mapping and exploration.
Such efficiencies can be gained by using small mother ship-deployed uncrewed vessels acting as relatively inexpensive mapping and sampling force multipliers or the use of small uncrewed vessels launched to from a mother ship to monitor and control autonomous underwater vehicles, allowing multiple operations simultaneously and “verified, directed sampling”, all while freeing the mother ship for independent operations.
We are also seeing the development of larger uncrewed vessels launched from shore with long-endurance and range, capable of carrying a full suite of deep ocean mapping and exploration tools.
All of these systems and approaches offer great hope but it is very early in our understanding of their full capabilities, costs and limitations and we must be careful not to overpromise, leading to disappointments and early abandonment of a potentially innovative approach, while at the same time maintaining the patience required to continue the research, investment and innovation that will hopefully bring us to a new world of efficient and effective ocean mapping and exploration that will allow us to meet our goal of complete coverage of the ocean.
1 Introduction
As we celebrate the 100th anniversary of The International Hydrographic Review (IHR) one might ask why we would devote a paper to the topic of the future of ocean exploration.
The answer is simple.
The mapping tools and techniques that have been pioneered, developed, and implemented by the hydrographic community are, without question, the primary tools of ocean exploration.
Almost all great explorations were built around the creation of maps that offered an initial geospatial context for “terra incognita”, and many of the great explorers were either mapmakers themselves or had mapmakers as key members of their team.
As early explorers looked outward to the seas, it was innovation in the development of navigation and positioning tools that allowed them to head far offshore and begin to map the extent of new landmasses and the resources they may possess.
Even in these earliest days of exploration there was the need to probe and chart seafloor depths to assure safe passage of the vessel as it approached unexplored regions.
Over the centuries that have passed since these early days of exploration we have, for the most part, completed mapping the distribution of terrestrial components of our planet and with modern satellite imaging technology, it is hard to imagine that there are new landmasses yet to be discovered.
Indeed, given the general availability of high-resolution satellite imagery and tools like Google Earth, almost anyone can produce a high-resolution image (and with overlapping imagery, a digital terrain model) or map of almost any landmass on earth, all precisely positioned through a Global Navigation Satellite System (GNSS).
As our knowledge and understanding of the distribution of the landmasses and peoples of our planet grew, the oceans became highways of trade.
The vessels that traveled these highways increased in size and capability, and the need for hydrographic mapping became, and still is, critical to assure the safe navigation of vessels in and out of ports (Jonas, 2023).
This evolution of mapping, exploration, discovery, technical innovation, and exploitation (of resources and sadly peoples), led to the remarkable growth of the global economy over the past few hundred years and the recognition that the ocean is vital in sustaining the lives and livelihoods of those who inhabit Earth.
As we have come into the 21st century, however, we have also recognized that this rapid growth has put tremendous strains on the health of the earth and that such growth cannot be sustained without a better understanding of its environmental impacts.
We have also learned that the earth is a complex system of interconnected systems (land, atmosphere, and ocean) and that understanding the ocean system, which represents more than approximately 71% of the earth’s surface and is central in regulating temperature, climate, CO , and other critical parameters, is key to developing approaches for the long-term sustainability of Earth.
It is this recognition of the key role that the ocean plays in feeding our planet as well as moderating and sustaining Earth’s climate and other environmental systems that has led to renewed emphasis on a better understanding the oceans as exemplified by the United Nations’ Decade of Ocean Science in Support of Sustainable Development1.
In developing the goals of the Decade of Ocean Science in Support of Sustainable Development, the complete mapping of the seafloor was recognized as a critical need yet, as we begin the U.N. Decade of Ocean Science, we do so with the sad recognition that at this point in time, less than 25% of the global ocean’s seafloor has been mapped and likely only about 5% of the global ocean volume has been explored.
How can we hope to understand the oceans we so depend on, when we do not yet know what is there?
In this paper we will explore the efforts being made to correct this situation and look at new technologies that might help us achieve the dream of a fully explored ocean.
Much of our focus will be on approaches to increasing the efficiency of mapping the seafloor, particularly the deep ocean seafloor beyond the realm of hydrographic organizations – because as was stated earlier, all exploration must start with the establishment of a geospatial context.
For this we will focus on the need to cover large, often totally unmapped regions rather than meeting critical hydrographic standards and thus much of the focus of this paper will be on new, more efficient ways to deploy critical ocean mapping and exploration sensors.
We will also look at new approaches for looking at the ocean volume and explore, in general terms, what the future might hold for the development and deployment of new tools that will support and enhance ocean exploration.
2 Mapping the global ocean seafloor
We begin our focus on ocean exploration by examining the state of our understanding of global seafloor bathymetry – a critical boundary condition for many ocean processes including controlling the pathways of deep-sea currents and the frictional loss of heat through generation of turbulence as currents pass over seafloor of various roughness.
Knowledge of both these parameters (the flow-path of deep ocean currents and seafloor roughness) is essential for the accurate modeling of global climate (Gille et al., 2004 and references therein).
In the polar regions, knowledge of where relatively warm subsurface currents can access margins of glaciers through bathymetric passages is essential to the modeling of glacial melting rates and the associated rise in global sea level (Jakobsson & Mayer, 2022).
Bathymetry is also essential for understanding deep sea hazards (slumping and other mass wasting events; Avdievitch & Coe, 2022) and for the prediction of storm surge and tsunami hazards (Parker, 2013).
Seafloor mapping is needed for establishing the routes of deep-sea cables and pipelines and has offered key insights into the nature of earth processes including the development of the theory of plate tectonics which was greatly aided by maps of seafloor morphology.
With the addition of backscatter, mapping has played a key role in delineating critical benthic habitats (Roberts et al., 2005).
Yet, as stated above, at the present time less than 25% of the seafloor has been directly mapped.
We say “directly mapped” because nearly complete global seafloor maps have been produced through the remarkable technique of deriving bathymetry from satellite altimetry (Smith & Sandwell, 1997).
Satellite-altimetry derived bathymetric maps are based on the principle that the sea surface is, to a first approximation, an equipotential surface of the earth’s gravity field that is affected by the gravitational attraction of large masses on the seafloor (e.g., seamounts) or the absence of mass (e.g., trenches) causing the sea surface to change elevation relative to the ellipsoid.
These changes in elevation can be measured by accurate satellite altimeters and thus the measured height of the sea surface reflects large-scale changes in bathymetry.
Through the comparison of satellite altimetry-derived measurements (for which there is continuous cover over most of the oceans) to actual soundings, Smith and Sandwell were able to predict the bathymetric values for places where there are no soundings.
This was a tremendous step forward in providing a global estimate of seafloor depths, however, the lateral resolution is on the order of 10–15 kms and the vertical accuracy is limited (Smith & Sandwell, 1997), meaning that to address many of the critical applications discussed above, the satellite altimetry-derived bathymetry does not have the needed resolution nor accuracy, and instead we must use direct measurements of depth.
Over the past 40 to 50 years, we have seen the technology used to make direct measurements of depth change radically.
For literally thousands of years depth measurements were made by measuring the length of a weighted line deployed from a vessel (lead line).
With the rapid evolution of sonar systems during the second world war, the single beam echo sounder became the tool of choice for most hydrographic and deep-sea ocean mapping missions.
Towards the end of the 20th century, multibeam sonars came out of classified military development and became commercially available.
Multibeam sonars (along with concomitant developments in satellite positioning and computer processing) revolutionized deep ocean mapping.
A single beam sonar (with a beam width of typically 15–30 degrees ensonifies an area on the seafloor with a diameter of approximately one half the water depth and produces only one depth (the shoalest depth) in the ensonified area with no knowledge of where that depth was within the area.
The position of the shoal can only be assumed to be directly below the vessel.
The result is a laterally averaged (over the beam footprint, i.e., half the water depth) portrayal of bathymetry.
Multibeam sonars, however, produce many narrow (depending on the model but typically 0.5 to 2 degree) beams in a broad (athwartship), but thin (alongship) swath across the seafloor perpendicular to the direction of travel of the vessel.
The result is many (tens to hundreds) of individual, highly accurate depth measurements across a swath that is typically three to five times the water depth wide.
With multibeam sonars thus came the ability to produce many individual high-resolution depth measurements from a single ping across a wide swath of the seafloor, revolutionizing our ability to map and visualize the deep ocean floor.
Because of vessel motion and the wide swath achievable, multibeam sonars require complex integration with other ships’ sensors (e.g., sound speed profiler, inertial motion sensor) but both sensor integration and post-processing software for these systems has improved greatly over the years and these systems have now been adopted by most institutions that map the seafloor.
High-frequency (200–400 kHz) shallow water systems (200 m or less) are small and compact (sonar array dimensions are on the order of 50 cm to 1 m), however, to maintain spatial resolution (narrow beam footprints), array sizes must scale up with the lower frequencies needed for deep-water mapping (12–30 kHz).
To achieve a 1-degree beamwidth at 12 kHz (the frequency used for full-ocean depth mapping) the sonar array must be on the order of 9 meters long.
Inasmuch as multibeam sonars use separate transmit and receive arrays (orthogonally oriented to create what is known as a Mills Cross or Mills T), if one wants to both transmit and receive 1degree beams, the receive array (which is mounted in the athwartship direction) must also be 9 m long, meaning that the vessel must be able to support a 9m long array along across its keel i.e., it must be a large vessel.
Thus, the state-of-the-art for deep-sea ocean exploration requires multibeam sonars mounted on large (typically 60 m or longer) survey vessels.
Recognizing the critical need for more complete maps of the ocean floor, the Nippon Foundation and GEBCO joined forces to create the Seabed 2030 Project, an ambitious effort aimed at establishing an infrastructure to facilitate the complete mapping of the global ocean floor by 2030.
The project was organized around four regional data centers (located in New Zealand, Germany, the U.S., and Sweden) and a global data center located at the British Oceanographic Data Center in the U.K.
The project began in earnest in 2017 with the data centers inventorying how much data was publicly available in global databases and addressing the difficult question of how much of the seafloor was mapped and at what resolution.
This analysis revealed that if the seafloor were divided into approximately 1 km square grid cells, 18% of them had at least one sounding in them and 9% of them had some multibeam data (Mayer et al., 2018).
Acknowledging that the beam footprint of a mapping sonar varies as function of depth, Seabed 2030 defined a series of depth-dependent grid resolutions for defining mapping coverage (see Mayer et al., 2018 for details).
When these depth dependent resolutions were considered, direct mapping data were available for only 6.9% of the seafloor.
Starting with this estimate of coverage in 2018, we have seen, through the concerted efforts of the Nippon Foundation-GEBCO Seabed 2030 teams and collaborating partners, the coverage increase to 24.4% at the beginning of 2023 (Fig. 1).
While this represents a tremendous increase in data availability, much of this increase was the result of discovery and provision of existing data sets and, as the amount of undiscovered data decreases, new data acquisition will be required to cover the more than 75% of the ocean floor unmapped by direct means.
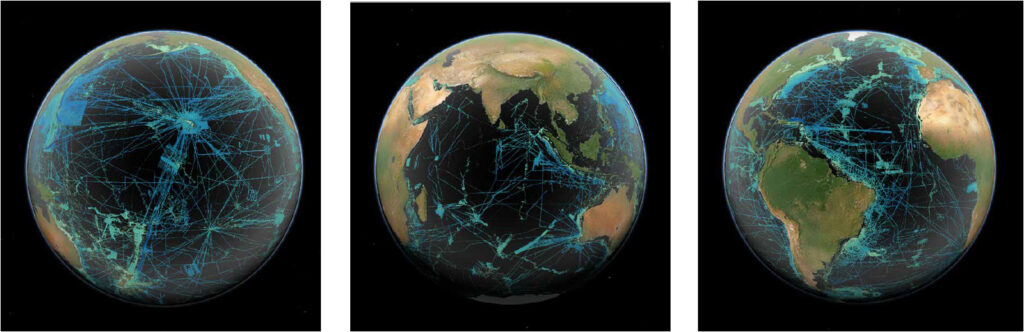
Fig. 1 Global bathymetric coverage in the GEBCO 2022 digital grid, representing most of the publicly available bathymetric data – viewer available at https://gis.ccom.unh.edu.
Areas that are black indicate no available direct bathymetric data.
Areas that are black indicate no available direct bathymetric data.
It has been estimated that it will take between 70,000 and 127,000 ship days to map, with state-of-the art multibeam sonar, the yet unmapped regions of the seafloor deeper than 200 m to the Seabed 2030 defined resolutions.
Given the high costs of operating the relatively large vessels needed to carry deep water multibeam sonars, the overall cost of this undertaking has been estimated to be on the order of three to five billion dollars (Mayer et al., 2018).This is a seemingly large number, but it should be noted that there have been many mapping missions to other planets that cost on the same order, raising the question of why we would not be willing to invest this amount in the mapping of our own planet.
While efforts continue to seek the funding to complete the mapping and exploration of the global ocean, here we will address whether new technologies can increase the efficiency of ocean mapping (and ocean exploration in general) and thus facilitate the complete mapping and extended exploration of our oceans.
3 Expanding the efficiency of ocean exploration with “autonomous” systems
Exploration of the surface of the earth (both land and oceans) was revolutionized with the advent of satellite imagery and remote sensing.
With respect to the oceans, these tools have offered tremendous insights into sea surface temperature, productivity, current and wave patterns.
Unfortunately, these insights are limited to the upper few meters of the ocean surface as the electromagnetic waves used for ocean imagery and remote sensing do not propagate far in water.
And thus, as outlined above, the fundamental tools for exploring the seafloor and volume of the ocean are acoustic systems, typically carried by large, slow, and expensive to operate, research vessels.
If we look at costs for typical sea-going research vessels we see that a significant percentage of the operating costs (in many cases over 50%) are associated with crew-related expenses (salary, travel, food, etc.).
Recognizing the significant crew-related costs involved in operating a research vessel, the concept of using uncrewed systems becomes an appealing option for increasing the efficiency of ocean mapping and exploration.
Note that we are calling these operations “uncrewed” rather than autonomous.
Despite the tremendous advances we have seen in the past few years in the development of uncrewed vessels, none of the current systems can be called truly autonomous; they all require some level of human interaction (pilots, operators, etc.) and thus do not remove all personnel expenses from the equation.
However, even at this early stage of development, the potential is there to demonstrate increased efficiencies.
We have already seen the private sector step up and demonstrate remote operation of small autonomous systems for near-shore surveys.
These are early days for these types of operations, but initial indications are that such approaches to survey work are enjoying success, demonstrating improved safety (i.e., no one offshore), 24 hour per day operation, and a reduced carbon footprint both with respect to the vessel and much-reduced travel requirements.
With respect to offshore ocean mapping and exploration, the use of uncrewed systems is in its infancy and much remains to be learned about concepts of operations and the ultimate viability and cost-effectiveness of operating uncrewed vessels in support of ocean exploration (Schmidt, 2020).
In addressing these questions, we will report on our own recent experiences working with funding from the U.S.
National Oceanic and Atmospheric Agency (NOAA) to explore the potential use of uncrewed systems in support of mapping and ocean exploration.
In relating these experiences, many will be based on the uncrewed vehicles that we are currently working with (DriX built by Exail and Saildrone) but will hopefully be generic enough to draw broader conclusions about the future role of uncrewed systems for deep ocean mapping and exploration.
4 Uncrewed systems as force multipliers for offshore mapping
One of the most obvious applications of uncrewed systems for offshore survey work is as a ‘force-multiplier’.
Large offshore research vessels typically have capitalization costs of over $100 million USD and day-rates often over $50,000 USD per day.
The diesel-powered uncrewed systems we have been using have capitalization costs of a few million dollars and operating dayrates (still being determined) on the order $10K per day (including operator costs).
Thus, the deployment of one or more uncrewed systems from a mother vessel offers the opportunity to multiply the efficiency of surveying for a fraction of the cost.
NOAA-funded work with DriX (Fig. 2) has demonstrated that this is indeed possible with the uncrewed vessel launched from the mother ship and surveying in tandem with the mother ship at speeds up to 10 knots and continuous operation over several days.
Full data transmission to the mother ship (typically at the end of each line) was possible when operating within the limits of the marine broadband radio system used to communicate between the mother ship and the uncrewed vessel (maximum about 20 km depending on atmospheric conditions).
In this mode, data processing can be done on board the mother ship as data is being collected, and data products produced not long after the survey is completed.
NOAA has estimated that such operations will increase survey efficiency by at least 40% (using a single uncrewed vessel).
Carrying more vessels should further increase efficiency but this has yet to be demonstrated.
To date the uncrewed systems that we have deployed from larger ships have been relatively small (7–8 m long) and capable of carrying high-resolution, shallow water multibeam sonars (200–400 kHz) along with broadband water-column mapping sonars (EK-80s), however, we have recently installed a new compact, 70–110 kHz multibeam sonar (EM-712) providing mapping capabilities to depths beyond 2000 meters and offering the option for using even our smaller uncrewed systems as force multipliers for deeper water mapping.
Depending on mission requirements, the uncrewed systems can be equipped with a range of sensors, including winch-deployed sensors (e.g., CTD) offering the opportunity to act as force multipliers for a range of oceanographic measurements.
At described above, at present, operation of uncrewed vessels can be carried out from a mother ship with full situational awareness and data transmission to ranges supported by marine broadband radio (~20 km).
Operations can be carried out beyond the range of the marine broadband radio (and even from shore-based facilities anywhere in the world) using satellite-based systems like Iridium’s Certus, however the bandwidth provided by these systems can currently can only support the transmission of situational awareness information and limited data rather than full data transmission.
The recent introduction of low-earth orbiting satellites supporting the maritime domain (e.g., Starlink) now offers the possibility of high-bandwidth communications with uncrewed systems and opens the possibility of full data transmission to and from uncrewed vehicles, world-wide.
These systems have just been introduced and their full capabilities and limitations have yet to be demonstrated but many efforts are currently underway to assess these systems including the recent installation of a Starlink system on our own uncrewed vehicles.
Testing of this system will begin over the next few months.
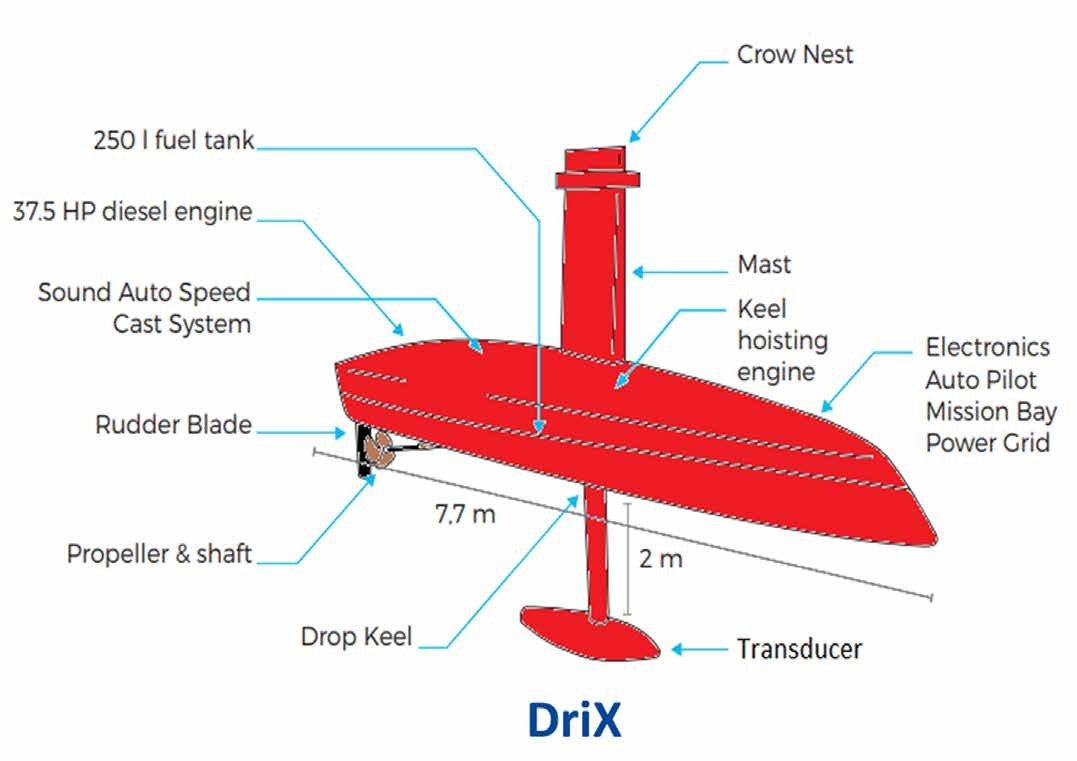
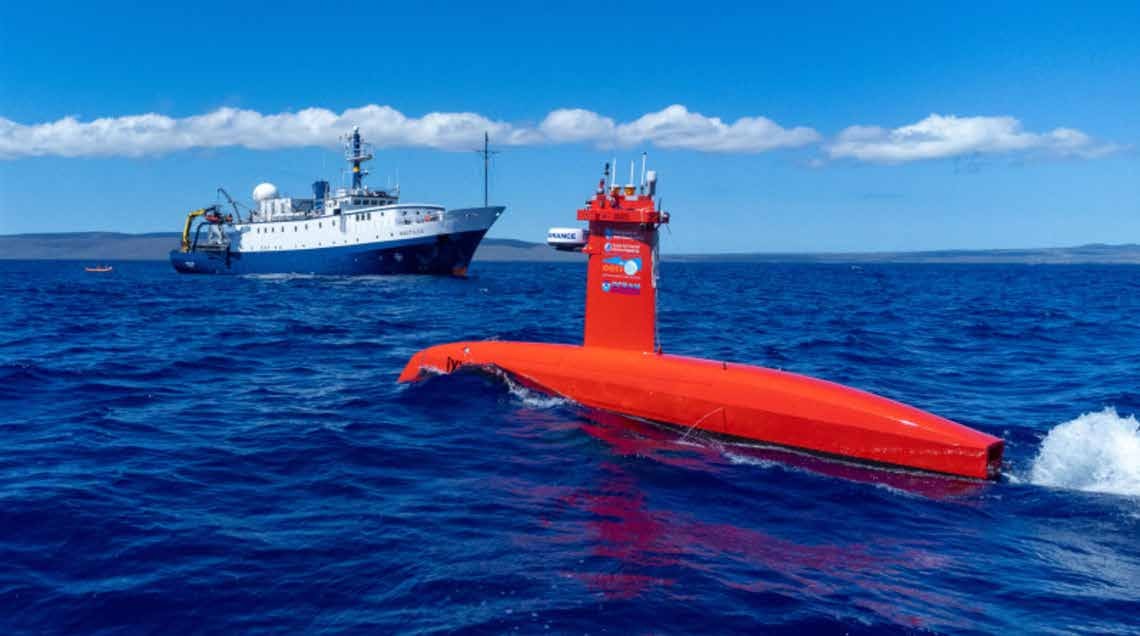
Fig. 2 The 7.7 m long DriX uncrewed vessel used by UNH as a platform to explore the applicability of uncrewed vessels to support ocean mapping and exploration missions.
5 Shore-deployed uncrewed systems for deep-water mapping and exploration
As we seek to extend the efficiency of deep-water mapping and other open-ocean exploration measurements, the size of the uncrewed vessel becomes an issue as the sonar arrays, winches, and wires, all need to be larger or longer to work in deeper water.
How large of an uncrewed vessel (or vessels) can be carried by a mother ship (and how large must the mother ship be)? At some point it becomes impossible to efficiently carry, deploy and recover uncrewed systems that are fully capable of undertaking deep water mapping and exploration activities and we must look to the concept of large, shore-deployed uncrewed vessels, capable of long-range deployments and carrying a full suite of ocean exploration tools.
A commercial entity, Ocean Infinity has been pioneering this approach through the development of a fleet of very large (78 m), fuel-efficient, offshore controlled vessels that will initially be crewed but are designed to eventually operate in an uncrewed mode.
Other manufacturers are also building larger platforms with extended range and the ability to carry deeper water exploration tools.
As these capabilities evolve and are implemented, the community will learn if these approaches prove to be a cost-effective and energy efficient approach to addressing our need to map and explore the global ocean, but the potential is clearly great.
As these purpose-built large uncrewed vessels are being built and approaches to autonomous operations being further developed, a concept to be explored is that of a mapping and exploration barge (Fig. 3) whose form-factor could accommodate a very large mapping sonar array (e.g., ~ 30m × 15m which would result in beamwidths of ~ 0.25 deg × 0.5 deg or a lateral resolution on the seafloor of on the order of 17.5 m × 35 m at nadir in 4000 m of water).
Such a platform would be relatively inexpensive to build (or acquire) and initially could be transported by a sea-going tug with a small crew at a fraction of the cost of a fully crewed research vessel.
The deck space available on a barge could accommodate a range of other ocean and atmospheric sensors providing a relatively inexpensive means of collecting a suite of ocean measurements (including sensors that need to be deployed by wire to probe the depths).
Sonar systems and other sensors could be operated remotely (the technology for this exists now) and with improved bandwidth from low earth orbiting satellites, these remote capabilities will increase.
As uncrewed ship operations evolve, we could envision a time when the entire barge could be a self-contained autonomous platform and a platform from which to launch other autonomous systems.
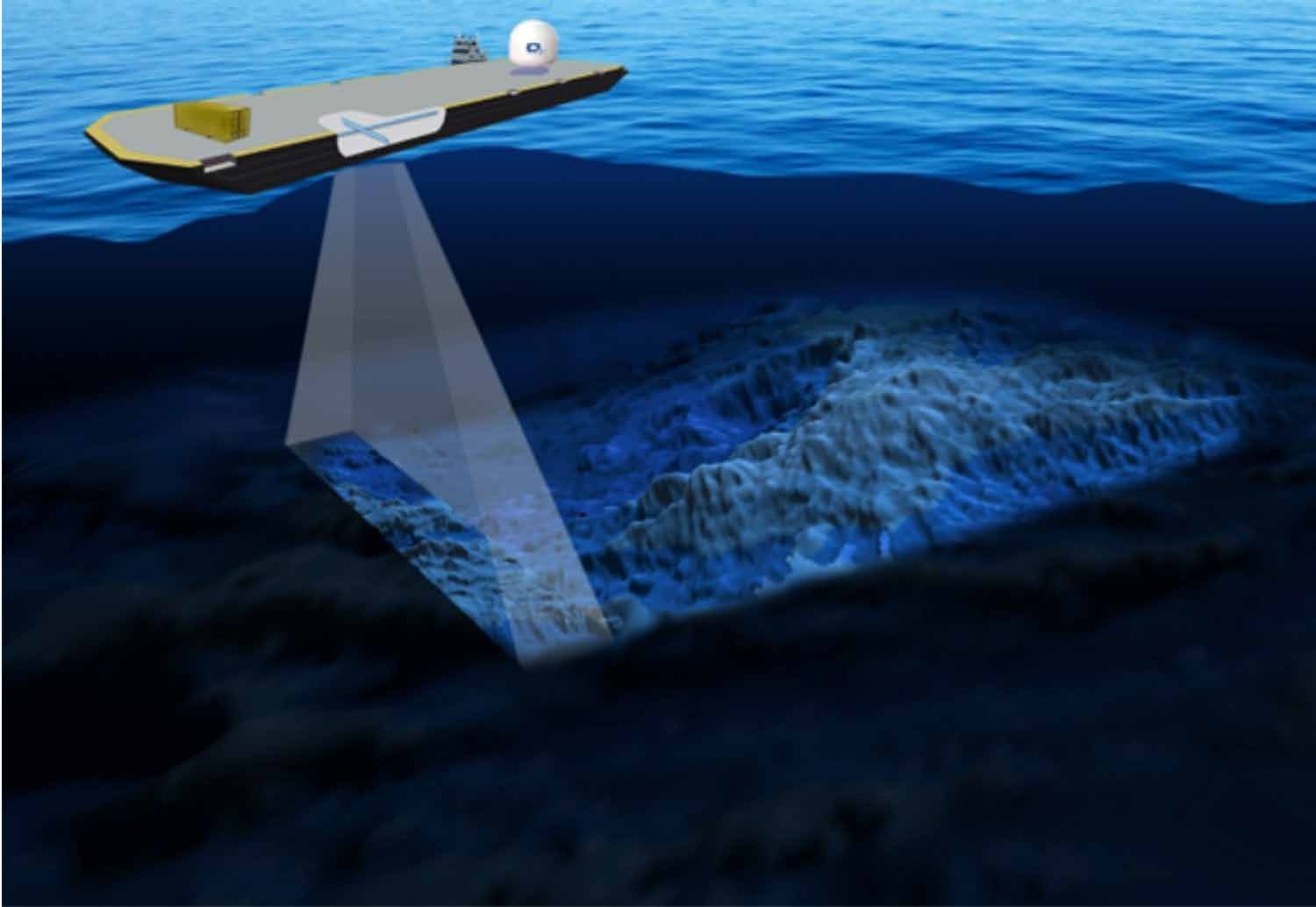
Fig. 3 The concept of an ocean exploration barge capable of carrying a very large MBES system and many other sensors.
Such a barge can be deployed with an ocean going tug at a fraction of the cost of a crewed research vessel and eventually could be designed to be a fully autonomous platform.
Image drawn by Ines Jakobsson.
Such a barge can be deployed with an ocean going tug at a fraction of the cost of a crewed research vessel and eventually could be designed to be a fully autonomous platform.
Image drawn by Ines Jakobsson.
6 Reducing fuel costs and extending range
The uncrewed vessels discussed above are all dependent on engines for their propulsion and while we are seeing exciting and important innovations in low-carbon footprint propulsion systems (i.e., hydrogen and ammonia-based), the dependence on any source of combustible fuel places limits on endurance of the vehicle.
The introduction of sail powered uncrewed systems has offered the intriguing possibility of mapping and exploration vessels with long endurance and a very low carbon footprint.
Several years ago Saildrone introduced a 7 m long, wind and solar powered uncrewed vehicle (Explorer) that has now demonstrated the ability to undertake long endurance ( >6 months), long-distance (>20,000 kms) missions surviving hurricanes and tropical storms.
These vehicles are equipped with an array of oceanographic and atmospheric sensors and have been equipped with single beam echo sounders, but they currently do not have the energy budget to support multibeam sonars.
Two larger vehicles are being introduced, however, the 10 m long Voyager and the 20 m long Surveyor (Fig. 4), each of which is equipped with an auxiliary diesel/electric power system that allows them to support multibeam sonars and winch-deployed sensors.
While the Voyager will be equipped with shallow water multibeam sonar systems, the 20 m Surveyor has already been deployed with a 30 kHz, EM-304 multibeam sonar, along with a shallow water EM-2040 and a full suite of EK-80 water column sonars creating a platform that is well-suited for deep-water ocean mapping and exploration.
Saildrones are operated from a shore-based control center with a sophisticated mission portal offering full situational awareness but not full data transmission.
With the advent of low earth orbiting satellites, full data transmission may be a possibility.
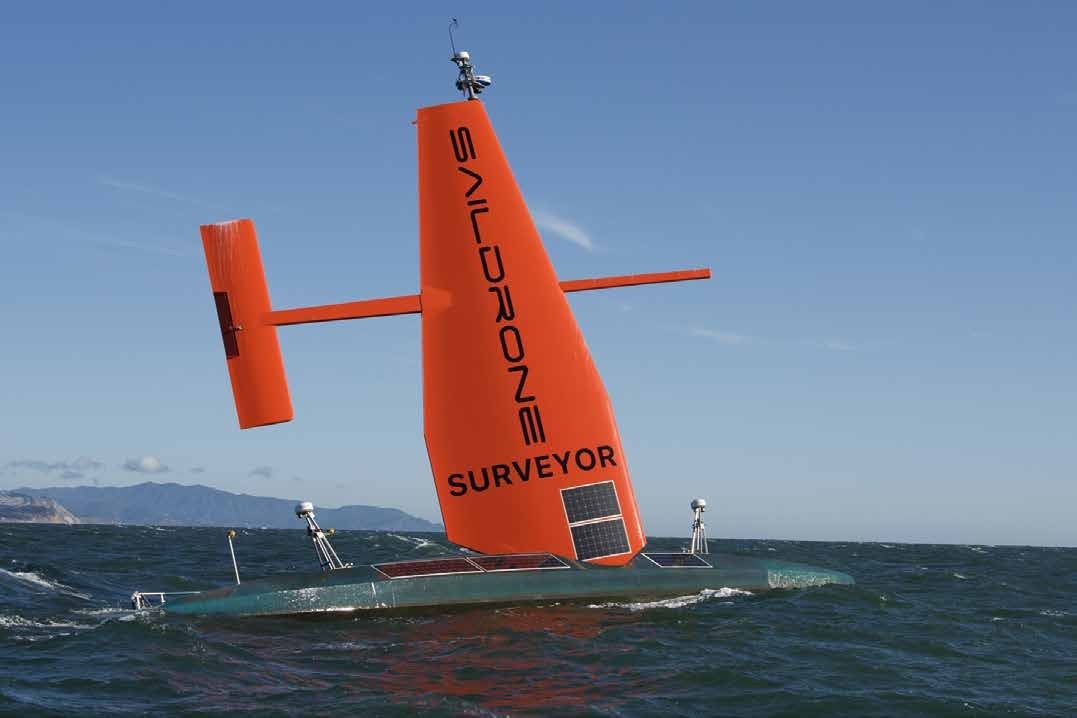
Fig. 4 The 20 m long Saildrone Surveyor arriving in Hawaii after a 28-day transit from San Francisco.
This prototype Saildrone Surveyor is equipped with a 30 kHz EM-304 and 200–400 kHz EM2040, a full suite of EK-80 broadband sonars and environmental sensors as well an ESP-3 eDNA sampling system from the Monterey Bay Aquarium Research Institute.
This prototype Saildrone Surveyor is equipped with a 30 kHz EM-304 and 200–400 kHz EM2040, a full suite of EK-80 broadband sonars and environmental sensors as well an ESP-3 eDNA sampling system from the Monterey Bay Aquarium Research Institute.
The Saildrone Surveyor underwent sea trials, funded by NOAA’s Ocean Exploration Program, in June of 2021 and then undertook its maiden voyage, an uncrewed mapping transit from San Francisco to Hawaii, funded by the Nippon Foundation/GEBCO Seabed 2030 Program, in July of 2021.
The Surveyor collected high-quality mapping data during this 3650km long transit, especially while under sail where the swath widened due to reduced noise (Fig. 5).
In the course of the transit, many lessons were learned about transit speed capabilities and how often the engine needed to be run in order to keep the batteries appropriately charged.
In 2022 the Saildrone Surveyor conducted work on behalf of NOAA’s Office of Ocean Exploration in remote areas of the Aleutian Islands (Fig. 6) and it is currently undertaking surveys of the U.S. EEZ off the coast of California.
As these surveys are completed, we continue to learn more about the capabilities and limitations of this innovative system particularly respect to endurance, speed, trade-offs between motoring and sailing, data quality, and overall cost-effectiveness of this approach to addressing our long-term mapping and exploration needs.
Saildrone is also using lessons learned to upgrade the design and produce a new generation of Surveyors with faster hull speed and extended capabilities.
Along with its acoustic systems, Saildrone Surveyor also carried a suite of environmental sensors including an exciting Environmental DNA (eDNA) sampling system from the Monterey Bay Aquarium Institute (MBARI).
eDNA is a relatively new approach of analyzing the nuclear or mitochondrial DNA that is released by organisms into water (Pillioid et al., 2013).
Analysis of eDNA allows the detection and identification of species that were present in the water mass and is a powerful new tool for understanding biodiversity.
MBARI has created an eDNA sampling system (ESP-3) that can be deployed on autonomous systems, filter water on command and preserves the samples for later analysis (Truelove et al., 2022).
On each of the completed Saildrone Surveyor missions, a complete suite of eDNA samples were taken with the ESP-3, demonstrating the viability of making these important environmental measurements from platforms much less expensive to operate than large, crewed research vessels as well as the potential of collecting these important measurements in remote and unexplored regions.
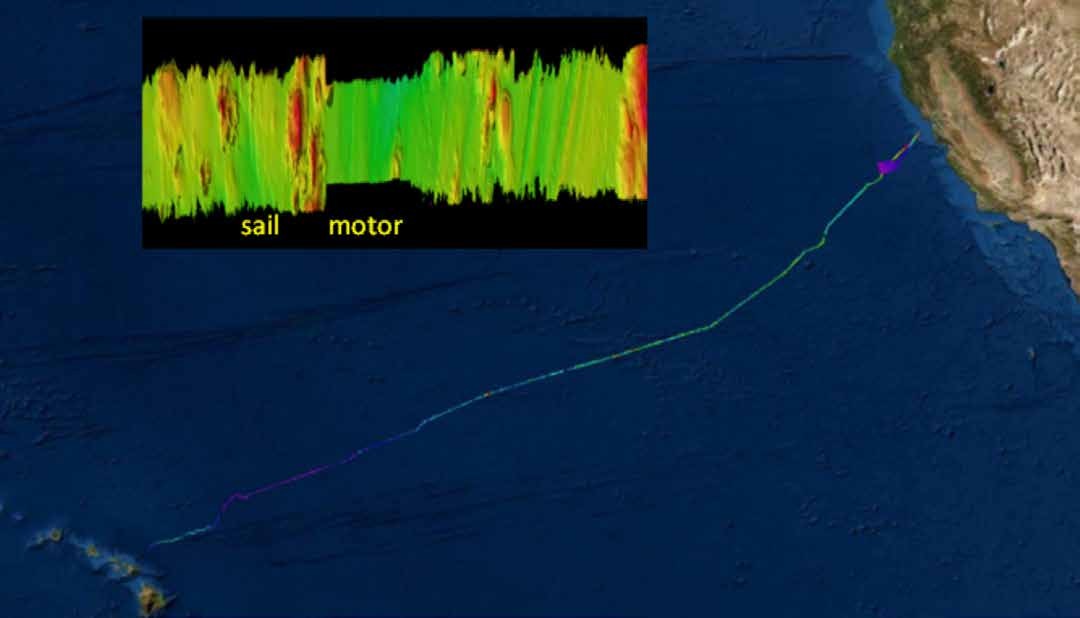
Fig. 5 The maiden voyage of the Saildrone Surveyor as it transited from San Francisco to Honolulu covering 3650 km over 28 days.
Insert shows increase in achievable swath width coverage going from using diesel motor to pure sailing mode.
Insert shows increase in achievable swath width coverage going from using diesel motor to pure sailing mode.
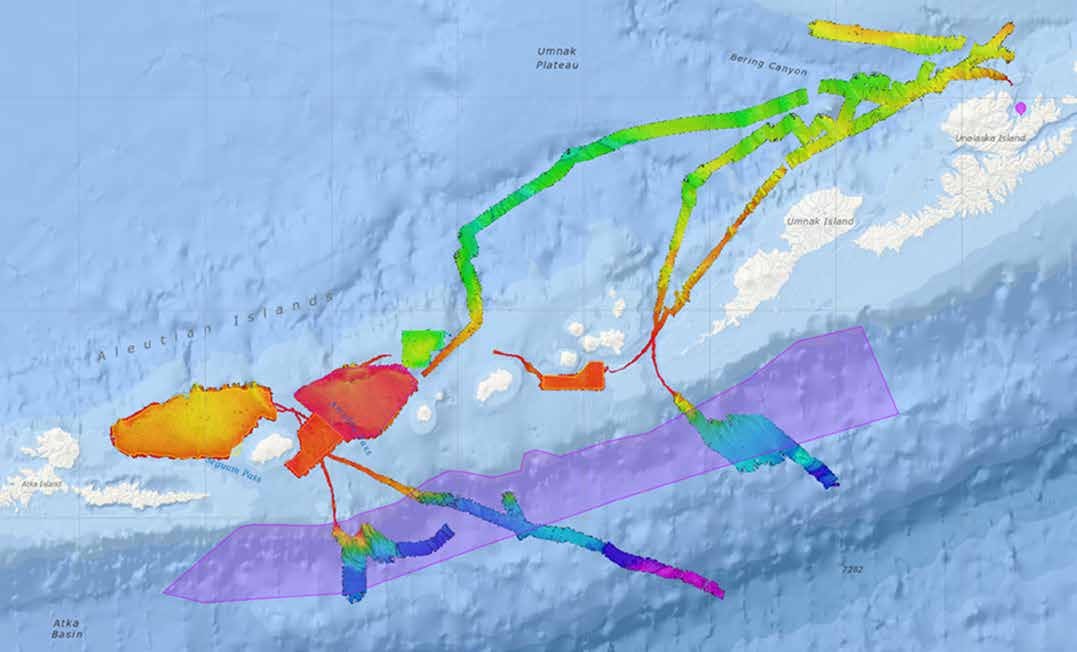
For this mission Saildrone departed from San Francisco, had a port call in Dutch Harbor and returned to San Francisco.
7 Every measurement counts
While our aspiration is to see the ocean completely mapped and explored with the greatest resolution possible, the unmapped and unexplored regions of the oceans are so vast that we must recognize that any additional measurement adds to our knowledge base.
ARGO floats are freely drifting floats that are deployed through an international program and designed to collect temperature, salinity, and pressure measurements over the upper 2000 m of the ocean.
The floats use a buoyancy engine to profile down to depth, drift (typically at 1000 m) for about 10 days, profile down to 2000 m, and then return to the surface to transmit their data via satellite.
They repeat this cycle until their batteries are depleted (typically about 3–5 years).
A new suite of floats can also collect biogeochemical measurements and some floats are being designed for deeper depths (to 6000 m).
There are currently about 4000 floats drifting around the oceans providing measurements of ocean temperature and salinity distribution that have revolutionized our understanding of ocean warming and other ocean processes.
With 4000 floats drifting around the ocean basins, it has been estimated that approximately 8% of these floats will ground in regions where bathymetry is poorly constrained and van Wijk et al.
(2022) have cleverly proposed that in these situations, information on grounding depth can supplement our bathymetric data base and provide additional bathymetric information in remote regions.
Taking the concept of using a profiling float for bathymetric data acquisition one step further, Seatrec Inc.
is developing, though funding from Schmidt Marine Technology Partners, the infiniTE™ (infinite Thermal Energy) float, a profiling float powered by an energy harvesting system that derives power from the thermal differences in the upper ocean (Fig. 7; Jones et al., 2011).
The energy harvesting system provides enough power for the profiling float to make as many as three profiles per day and to use an active echo-sounder to collect bathymetric information along with the standard oceanographic data collected with a CTD.
Like Argo floats, the infiniTE floats are navigated by GPS and transmit their data via satellite link when they surface (Coley, 2023).
The first two prototypes of these new echo-sounding floats will be deployed for the first time in the spring of 2023.
Should these floats prove successful, they offer a relatively inexpensive approach for long-term bathymetric data collection in remote regions of the oceans where ships rarely venture and where existing data coverage is very sparse.
Beyond their ability to provide single beam sounding measurements in remote regions, it may be possible to use a group of profiling and echo-sounding floats to form a large baseline sparse array that can make very high-resolution bathymetric measurements over a wide area (Ryu et al., submitted).
Envision an array of echo-sounding infiniTE floats deployed over an area of say 20–40 km.
If the floats are commanded to profile at the same time, they can capture the three-dimensional sound speed field of the ocean volume.
When the floats return to the surface, several of the floats could be used as acoustic sources and the rest used as a disaggregated sparse array of receivers, all precisely positioned with GNSS.
The combination of the precise location of sources and receivers along with knowledge of the three-dimensional sound speed field offer the opportunity to solve the more difficult aspects of providing a coherent 3-D solution for the ray paths leading to the possibility of very high-resolution bathymetric solutions over a wide area.
Certainly speculative, but research efforts are currently underway at MIT Lincoln Labs to explore the use of sparse arrays for bathymetric measurements.
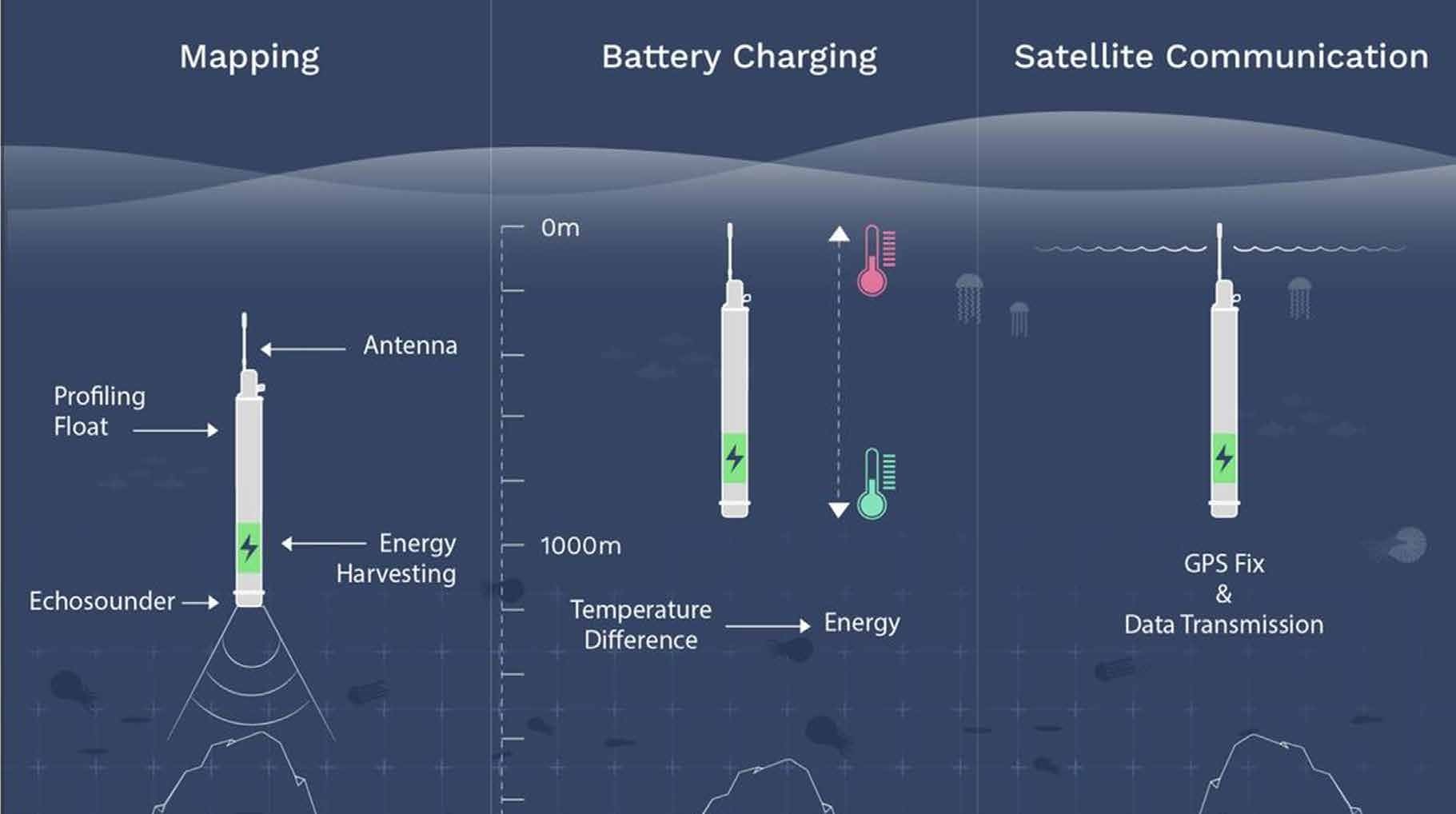
The profiler will dive to depth and make a sounding then drift and make another sounding before returning to surface getting another GPS fix and transmitting data via satellite.
Image courtesy of of Yi Chao, Seatrec Inc.
While we have emphasized the role of uncrewed systems as a force multiplier that has the potential to greatly increase the efficiency with which we can map and explore the oceans by providing more cost-effective and fuel-efficient means to cover large areas of the oceans, there is another important role that uncrewed vehicles can play in enhancing our ocean exploration capabilities.
Ocean exploration missions often begin with regional mapping to set the geospatial context before more detailed exploration and prosecution of targets takes place.
Regional mapping is indeed a role where we have argued that uncrewed systems can provide increased efficiency by covering large areas at reduced cost with respect to ship time, personnel, and fuel.
But once targets are selected for detailed mapping and exploration (e.g., a hydrothermal vent field), a large mother ship typically deploys high-resolution mapping and imagery systems (e.g., ROVs and AUVs) to provide detailed analyses and sampling.
Typically such operations involve the deployment of a single system at a time and require the dedicated support of the large mother vessel.
We have recently, however, demonstrated how, through the use of relatively small uncrewed systems, multiple underwater uncrewed vehicle operations can be supported and the mother ship freed to pursue independent activities.
On a recent expedition of the E/V NAUTILUS (NA-139; May 2022), sponsored by NOAA’s Office of Ocean Exploration, teams from the University of New Hampshire, the Woods Hole Oceanographic Institution and the Ocean Exploration Trust, worked together to demonstrate collaborative behaviors among multiple vehicles and the mother ship (NAUTILUS) to demonstrate the potential for simultaneous, multiple vehicle deployments, and thus increased efficiency in the use of these expensive assets.
On board NAUTILUS was the 7 m long DriX uncrewed system described earlier, the Mesobot, a slow moving autonomous underwater vehicle designed to track particles in the mesopelagic (midwater) and collect eDNA samples (Yoerger et al., 2018), and NUI, a hybrid ROV/AUV designed to travel 10s of kms laterally underwater connected to the mother ship by a thin fiber but then act as an AUV when the fiber is cut (Barker et al., 2020; Fig.8).
NUI carries a full suite of cameras and acoustic systems as well as a manipulator arm for sampling.
The initial step in developing collaborative behaviors was to establish a common acoustics communication system among the vehicles.
This was achieved through the use of a Sonardyne HPT 3000 tracking and communications transceiver mounted on the DriX and acoustic transponders mounted on the AUVs.
The team also developed a series of standard Robot Operating System (ROS) messages to allow the transfer of data and commands among the vehicles.
Once deployed, the DriX was able to track, communicate and follow the AUVs using the HPT 3000.
Inasmuch as the AUVs move very slowly (1–2 knots) and DriX tends to travel much faster, a special behavior was created to allow DriX to circle above the vehicles and thus always stay centered above the vehicles (Fig.9).
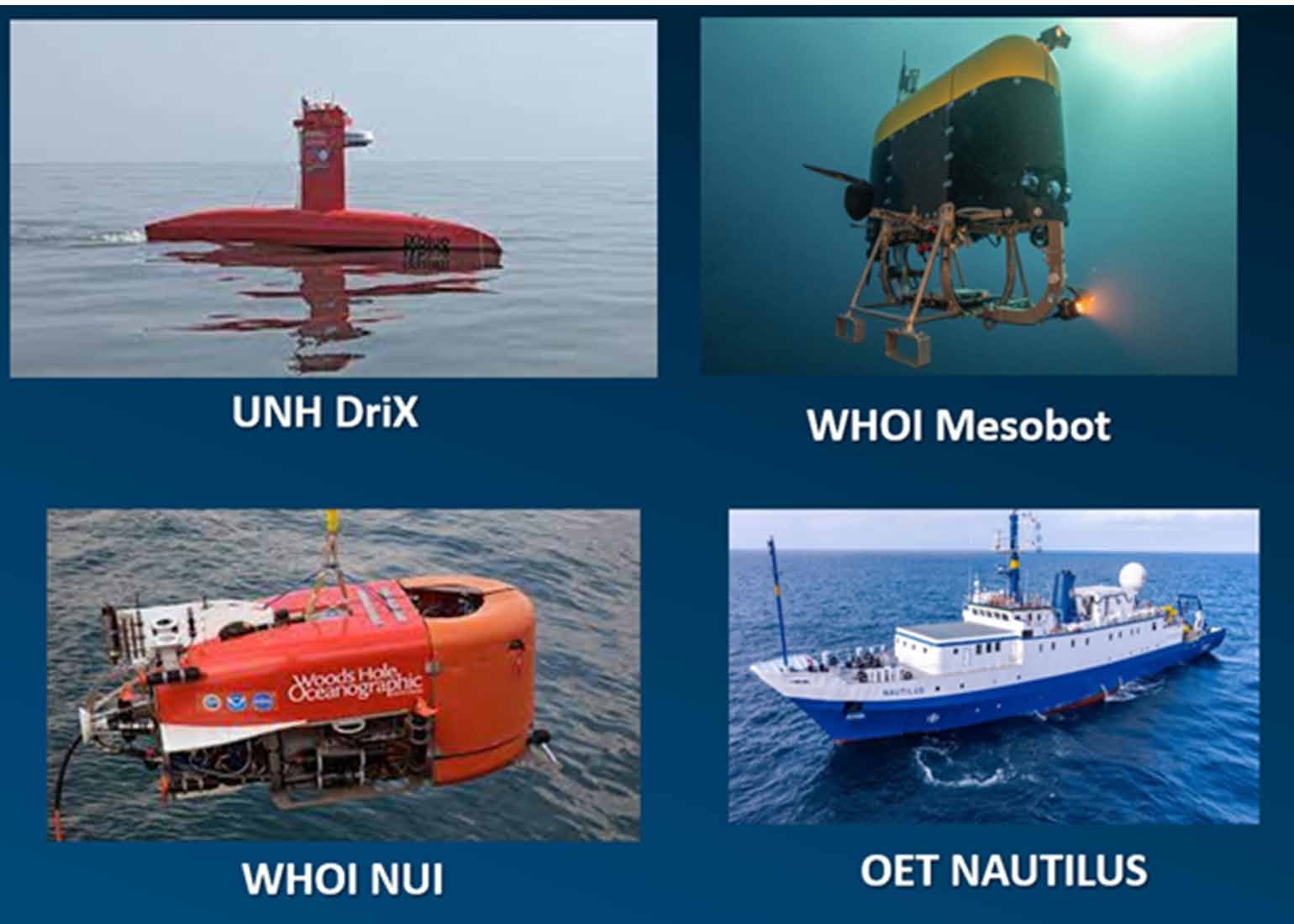
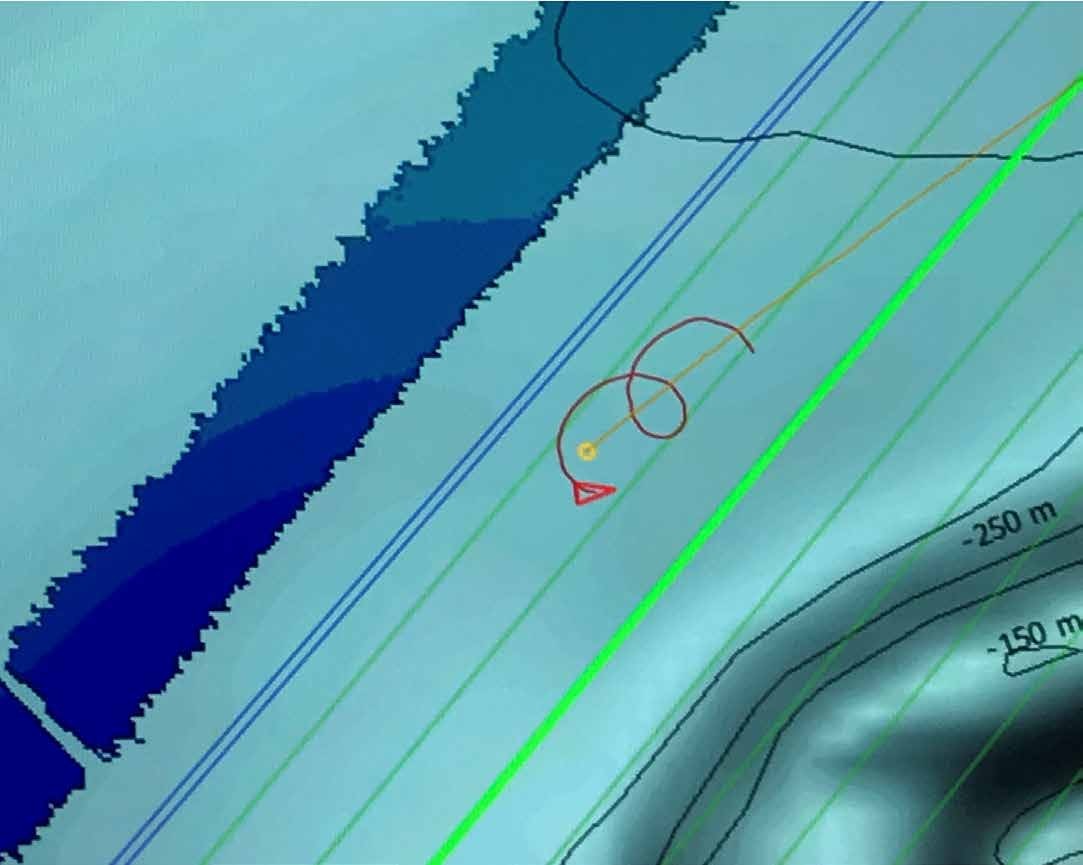
Full situational awareness of the position and status of all vehicles was provided by CAMP – CCOM Autonomous Mission Planner (Arsenault & Schmidt, 2020), an application that serves as a backseat driver for autonomous vehicles and allows for monitoring the progress of multiple vehicles and the mother ship as well as the ability to quickly and easy make and revise mission plans, all on top of raster images (e.g. charts or bathymetry; Fig.10).
Most importantly, the sonars on the DriX were able to provide real-time (while within range of the marine broadband radio – ~20 km) information on potential targets for investigation by the AUVs.
For example, while Mesobot was deployed, the EK80 on the DriX which was following, tracking, and communicating with Mesobot, located a strong midwater scattering layer that was seen in real-time through data transmitted from DriX to the mother ship (Fig.11).
Commands were sent to Mesobot to move to the layer and when Mesobot was in the layer (visually confirmed by the ability to see Mesobot in the EK-80 display), Mesobot was sent commands to begin sampling for eDNA (Fig.12).
This ability to see, in real-time, the location of the AUV and its sampling systems with respect to the target layer opens a new world of “verified directed sampling”.
This ability will remove many ambiguities and uncertainties associated with the sampling or measurement of oceanographic phenomena as well as help address questions of sample bias and avoidance.
Through the combination of surface and subsurface autonomous vehicles we will be able to see exactly where samples or measurements are being taken and how the targets being sampled behave during sampling.
With respect to gained efficiencies, we were able to operate with Mesobot, NUI and DriX simultaneously, with DriX on the surface, Mesobot exploring the midwater, and NUI mapping and exploring the seafloor.
Throughout this exercise, the DriX served as the communications link between the vehicles and the mother ship, tracking their location and transmitting data and commands to and from the ship.
When NUI went into full AUV mode (i.e., off the fiber tether), the NAUTILUS was free to leave the direct vicinity of the AUVs and carry on its own activities.
During this mission we were limited by the range of the marine broadband radio and thus the NAUTILUS could not go beyond about 20 km from the AUVs’ operating area but with the implementation of low earth orbiting satellite communications from the uncrewed surface vehicle, there should be no limit to the range at which the uncrewed surface vessel could operate from the mother ship while following the AUVs, and thus the AUVs could easily be monitored and controlled from a shore-based facility anywhere in the world.
Carrying this one step further, as demonstrated in the Shell Ocean Discovery XPrize competition, we are already seeing the introduction of large-format shore-based uncrewed surface vessels that can carry and deploy their own ROV (Zwolak et al., 2020).
With such systems we can envision the uncrewed surface vessel using its suite of sensors for broad exploration and initial mapping and when targets of interest are found (perhaps by automated processing and detection algorithms on board the vessel), the ROV can then be deployed for detailed study and mapping of the targets without human intervention.
With the advent of high-bandwidth communications through low-earth orbiting satellites in combination with growing capacity for telepresence (Raineault et al., 2018) shore-based scientists can remain in the decision and control loop offering tremendous opportunities for truly expanding the footprint of ocean exploration while bringing the wonders of deep-sea exploration to school children and the public at large, world-wide.
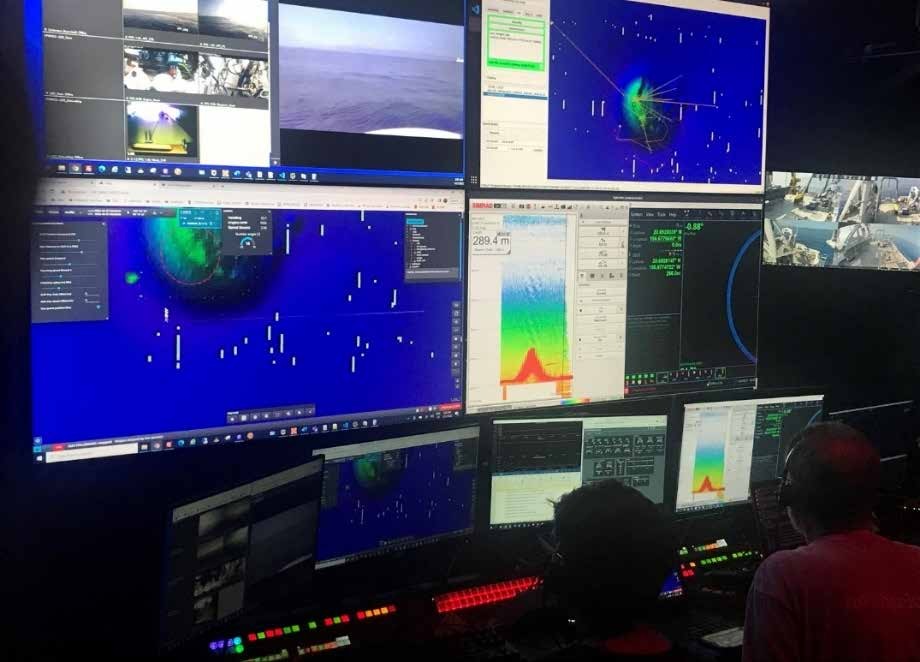
Fig. 10 The UNH CAMP applications providing full situational awareness of the location of multiple vehicles in the context of underlying raster mapping data.
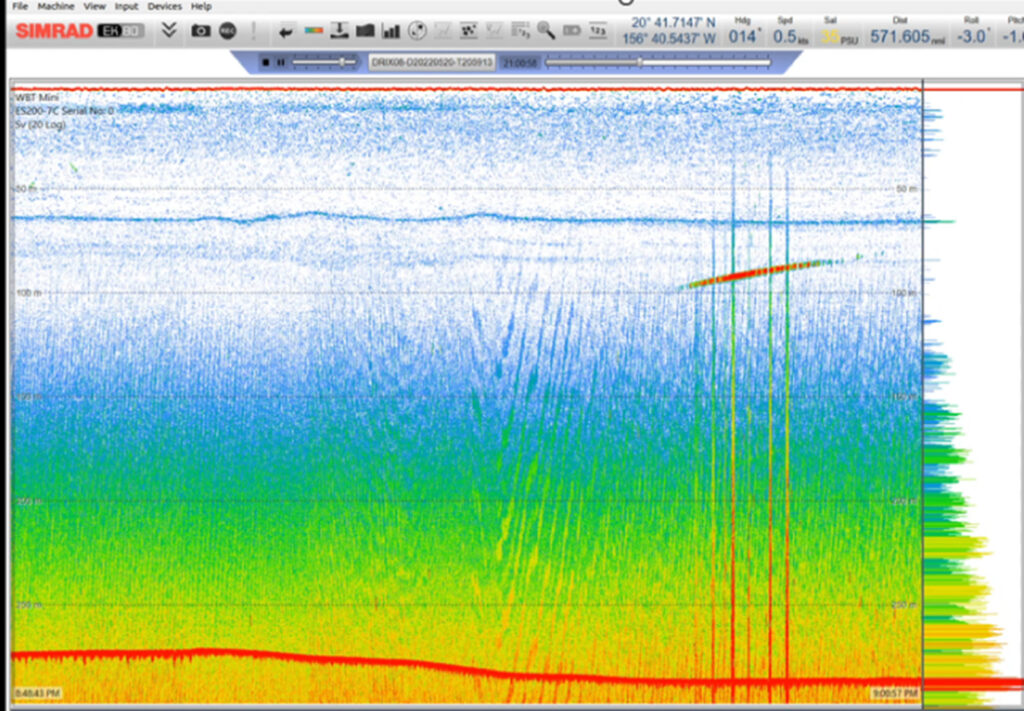
The red diagonal line is the reflection of Mesobot traveling underneath DriX as it is being directed by commands from the mother ship to move to the scattering layer.
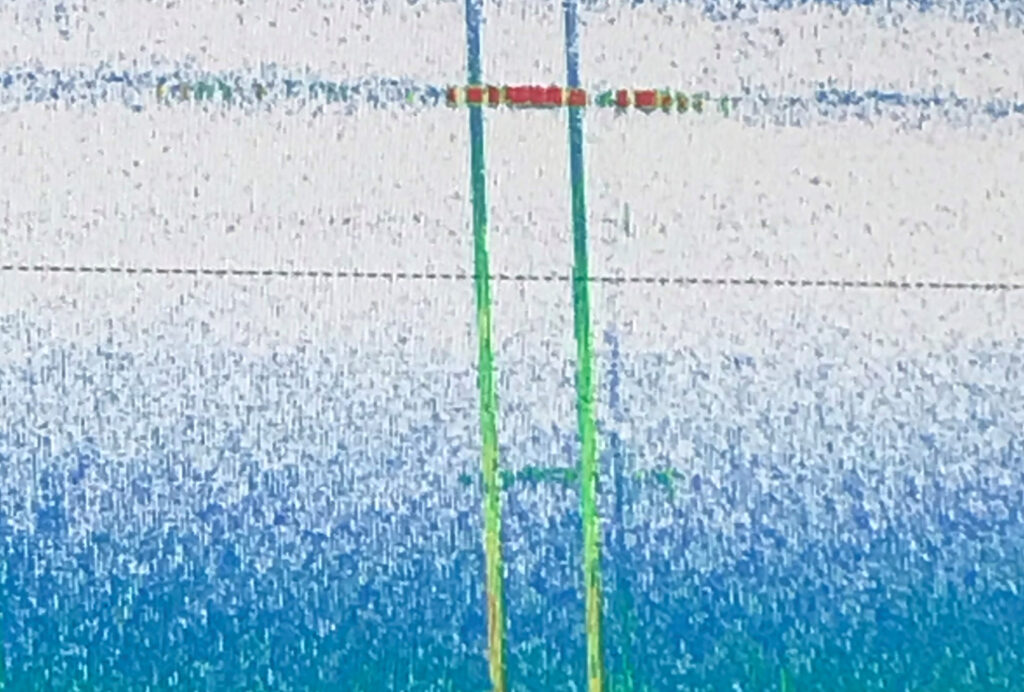
9 Conclusions
The global community is recognizing that the long-term sustainability of humankind is inextricably linked to our understanding of the oceans and the processes within them that control the distribution of heat, CO, nutrients, and other critical parameters.
Yet our oceans are vast and despite more than 100 years of effort, our traditional approaches to studying the oceans using large, crewed research vessels, have resulted in less than 25% of the seafloor mapped and on the order of only 5% of the ocean volume explored.
If we are to address the pressing problems associated with climate change, sea level rise, and many other ocean-related issues in a timely fashion, we will need to accelerate our efforts to map and explore the deep global ocean as we cannot understand what we do not know.
Uncrewed surface vessels are being developed that may offer the chance to greatly reduce the cost and increase the efficiency of global mapping and exploration.
Multiple approaches are being taken.
The deployment of relatively small uncrewed vessels that can be carried from larger mother ships has already been demonstrated as a force multiplier offshore, but there are limits to the size of the uncrewed vessels that can be carried aboard a mother ship and this limits the size of the sensor systems that can be carried (and thus the water depths that can be mapped and explored.) Additionally, the range over which full data sets can be transmitted from the uncrewed vessel to (and from) the mother ship has been limited by the capabilities of marine broadband radios (typically on the order of 20 km depending on atmospheric conditions), but with advent of high bandwidth transmission through low-earth orbiting satellites, we may be able to operate uncrewed vessels (and transmit full data sets) much further from the mother ship or from shore-based stations anywhere in the world.
Uncrewed vessels can also play an important role as command-and-control stations, monitoring and controlling the location and behavior of underwater autonomous systems and relaying data and commands from the mother ship or shore stations to underwater vehicles, while freeing up the mother ship to pursue independent tasks.
The ability for operators to see, in real-time through the uncrewed surface vessel link, the location of underwater vehicles with respect to seafloor or midwater targets combined with the ability to direct the underwater vehicle to those targets, opens a new world of verified directed sampling where there is no ambiguity about what has been sampled or imaged.
Larger uncrewed vessels are being developed that can be launched from shore, with ranges of many 1000s of kms, that can carry deep-water sonars and exploration tools, and can spend extended time offshore.
These systems are exploring the use of energy efficient fuel systems and some can deploy their own remotely operated vehicles.
Large uncrewed sail-powered vessels are also being developed that offer a very low carbon footprint, the ability to carry large mapping systems and other sensors and the potential for long deployments to remote regions.
It is clear that much is going on with respect to the use of uncrewed surface vessels for ocean exploration and mapping, however, the critical words in the paragraphs and pages above are “being developed”, “may be able to”, “have the potential to”, and “exploring the use of”.
We are at the very early stages of understanding just what role uncrewed and eventually truly autonomous systems will play in our quest to map and explore the oceans.
We need to fully understand the capabilities, limitations, and tradeoffs associated with these systems with respect to gained efficiencies and overall costs.
The great challenges we face with respect to mapping and exploring the oceans impel us to seek new technologies and there is great potential that uncrewed systems will revolutionize our efforts, but the change will not be instantaneous and will not come without continued research, investment, innovation, and the steep learning curves associated with technological revolutions.
We must find the balance between too quickly jumping into approaches that are really not mature enough to appropriately serve our needs and thus lead to disappointment and abandonment of what might eventually be a very effective tool, and the frustration associated with long-term research and development projects.
The broad recognition of the great potential of uncrewed vessels across the private sector, government agencies, academia and NGOs, and the collaborations being established among these organizations is helping us find this balance and will hopefully expeditiously bring us to our collective objective of much more efficient mapping and exploration of the world’s oceans.
Links :
- GeoGarage blog : 3 weeks, 15 unmanned systems: Navy launches 'Digital ...
NYTimes : The tiny craft mapping superstorms at sea
ReplyDeleteNYTimes : The hurricane and the Saildrone
ReplyDelete